User:Adam Mirando/Sandbox 1
From Proteopedia
|
Contents |
Overview
Xanthine oxidoreductase (XOR) is an extensively studied metalloflavoprotein from the molybdenum hydroxylase family that is found in a variety of different organisms, ranging from bacteria to eukaryotes [1]. XORs are dimeric enzymes typically around 280 kDa in size with two interconvertible forms: xanthine dehydrogenase (XDH) [1.17.1.4] and xanthine oxidase (XO) [1.17.3.2]. Conversion between the two forms is mediated through the reversible oxidation of several cysteine residues or irreversible trypsin truncation [2]. XOR is involved in purine catabolism, catalyzing the oxidation of hypoxanthine and xanthine to urate through the extraction of two electrons [3]. The transport of these electrons is facilitated by the molybdenum of the , two , and a bound coenzyme. In XDH the electrons are then passed preferentially from the reduced flavin to a final NAD+ acceptor, creating NADH [4]. Apart from NADH, XDH may also use O2 as a final electron acceptor. In contrast, conversion to the XO form precludes NAD+ from binding, permitting only the use of O2. The reduction of O2 produces substantial amounts of H2O2 and superoxide as byproducts [3][5]. The products of these enzymes have been implicated in the innate immune response as a balancer of redox potential and antioxidant (urate) provider[6] and cardiovascular disease, such as atherosclerosis [7], ischemia-reperfusion injury, and chronic heart failure [8] [9].
Structure
|
Bovine xanthine dehydrogenase has the overall dimensions 155 Ǻ x 90 Ǻ x 70 Ǻ in its dimeric form and 100 Ǻ x 90 Ǻ x 70 Ǻ for the individual protomers. The overall structure of the enzyme can be categorized into three key domains. The (green, residues 1- 165) harbors the two Fe-S clusters (shown in yellow). The second, (blue, residues 226-531) contains the FAD domain (shown in orange) and the NAD+/O2 binding site. The (purple, residues 590-1332) contains the molybdopterin cofactor (shown in red) and is positioned close to the interface between the other two domains. This structure allows for interactions between co-factors of the same protomer. However, closest distance of co-factors between the two subunits is greater than 50 Ǻ, suggesting that the two subunits do not cross communicate [2].
Xanthine Dehydrogenase/Xanthine Oxidase Conversion
Xanthine oxidoreductase has two functional forms: xanthine dehydrogenase and xanthine oxidase. This conversion is controlled by the oxidation state of Cys535, Cys992, Cys1316, and Cys1324. When these residues are reduced, the enzyme functions as a dehydrogenase, using NAD+ as its final receptor. Following chemical modification (ie fluorodinitrobenzene) or oxidation (ie 4,4'-dithiopyridine) the oxidase form is favored. Once oxidized, incubation with a reducing agent (ie dithiothreitol) will restore the enzyme to the the dehydrogenase form [5]. Studies involving the C535A/C992R/C1316S triple mutant, however, were unable to convert to the oxidase form. Consequently, crystal structures of this mutant revealed a monomeric structure, in contrast to the normally homodimeric wild type enzyme [5]. The mechanism of this conversion is thought to be the formation of disulfide bridges between Cys535 and Cys992 and Cys1317 and Cys1325[3][5]. Crystallographic data have show that residues 535 and 992 are capable of forming disulfide bonds[3]. Due to a distance of 15.7 Ǻ between the α-carbons of Cys535 and 992, the formation of a bond would require a substantial conformational change [2]. Further structural analyses reveal a peptide cluster composed of Arg426, Arg334, Trp335, and Phe549 that are tightly packed in the XDH but dispersed following disulfide bond formation. This modification is then transmitted to a loop consisting of residues 422-432 in the FAD domain restricting NAD+ from binding while opening a channel accessible for O2 in the now dispersed peptide cluster[3]. As a consequence of the loop shifting, Asp429 interactions with the flavin are eliminated while new interactions between the flavin and Arg426 are introduced. These new electrostatic interactions modify the flavin potential in favor of oxidase functiondomain[2] ( and : shown are Cys992 (red), Lys537 (cyan, XDH only, Cys535 could not be solved in either structure), peptide cluster (teal), Arg426 (blue), Asp529 (yellow), 422-432 loop (fuchsia), and FAD (silver). Similar effects are also noticed in the case of irreversible conversion to XO by trypsin digestion after Lys551. The cleavage disrupts an interaction between Phe549 and Arg427 resulting in a shift of the 422-432 loop and an exchange of Asp429 for Arg426 [2]. The oxidation Cys1317 and 1325 also eliminates the NAD+ binding properties of XOR. The two residues are 20.5 Ǻ on the C-terminal tail of the enzyme. The insertion of this tail into the FAD domain appears to be essential for the binding of NAD+. Oxidation of the cysteines changes the structure of this loop, preventing its insertion into the FAD domain[5] (: shown are Cys1317 (fuchsia), Cys1325 (green), and the C-terminal tail (yellow); C-terminal tail not solved in XO structure).
Mechanism
Substrate Binding and Intermediate Stabilization
|
Several active site residues have been implicated in the substrate binding and catalytic roles of xanthine oxidoreductase. The molybdenum center is accessible only through a 5 Ǻ x 3 Ǻ channel that is 5 Ǻ deep. The active site pocket itself is lined by several : Glu802, Leu873, Arg880, Phe914, Phe1009, and Glu1261 [10][11]. The several hydrophobic residues, Leu873, Phe914, and Phe1009, serve to form the active site pocket[10][11]. The conserved Glu1261 [3] is located near the molybdopterin co-factor (see “Xanthine Oxidation Mechanism” above”) and acts as a general base to extract a proton from the hydroxyl group of the molybdenum center. The complete loss of enzymatic activity following mutations of this residue confirms its important role in catalysis [12]. Arg880 and Glu802 are thought to be involved in the mechanism through the formation of stabilizing interactions with the reaction intermediates [3][10][12].
The interactions of Arg880 and Glu802 appear to vary with analogous substrates and inhibitors, leading to the development of two different modes of substrate binding in the case of the xanthine oxidation mechanism. One mechanism () suggests that Glu802 forms hydrogen bond interactions with the C6 carbonyl and N7 of xanthine while Arg880 forms hydrogen bonds with the carbonyl of C2 [3]. The second mechanism () suggests an inverted orientation of the substrate allowing for hydrogen bond interactions between Arg880 and the C6 carbonyl of xanthine. In addition to the facilitation of substrate binding, this orientation would allow for a more catalytic role of Arg880, in that it would allow for stabilization of the enolate intermediate [3][10]. Crystal structures involving the alloxanthine inhibitor are indicative of scheme 1. However, crystal structures using the inactive, desulfinated enzyme in the presence of xanthine are supportive of scheme 2 [10]. In further support of scheme 2, Arg880 to methionine (R880M) mutants exhibit a complete loss xanthine activity. In contrast, Glu803 to valine (E802V) mutants show only a reduction in activity corresponding to an 8-fold increase in Km and a 92.6% reduction in kcat compared to the wild type enzyme [12]. The hypoxanthine oxidation mechanism, however, shows an opposite response to the mutations. The E802V mutants exhibit a complete loss of activity while R880M show only a reduction (12-fold increase in Km and a 98.9% reduction in kcat). As such, a hypothetical binding arrangement in which Glu802 forms hydrogen bond interactions with the C6 carbonyl and N1 of hypoxanthine has been suggested [12].
Xanthine Oxidation Mechanism
Several mechanisms have been suggested for the oxidation of xanthine to urate by xanthine oxidoreductase. However, a substantial amount of data appears to favor a mechanism in which a deprotonated molybdenum hydroxyl attacks the C8 atom of xanthine. This mechanism begins with the extraction of a proton from the hydroxyl of the molybdenum center by Glu1261 [13], an event computed to occur readily in the presence of the substrate [14]. The electrons from the deprotonated oxygen are then free to attack the electrophilic C8 atom of the bound . The formation of glutamic acid stabilizes this structure through hydrogen bond interactions with the N1 atom [15]. Crystalographic data has also suggested possible stabilizing interactions between Arg880 of the active site and enolate tautomerization at C6 [10]. Bond formation between the substrate and the molybdenum center orients a Mo = S moiety equatorially to the substrate, positioning it favorably for a concomitant hydride transfer from xanthine N7 [3]. Extraction of this hydride produces Mo-SH and reduces the Mo center from Mo VI to Mo IV. This intermediate breaks down through electron transfer from the molybdenum center through the iron-sulfur clusters, known as Fe-S I and Fe-S II to the bound FAD, forming FADH2. In this mechanism the Fe-S clusters function as electron sinks, maintaining an oxidized Mo-cofactor and a reduced FADH2. The Mo atom serves as a transducer between the two electrons passed from the substrate to the single electron of system of the Fe-S clusters. The transfer of electrons can be monitored through the formation of the paramagnetic transient Mo V [16]. Subsequent reduction of NAD+ to NADH in the case of xanthine dehydrogenases and O2 to H2O2 and superoxide for the oxidase regenerates the oxidized FAD. Other mechanisms involving protonated molybdenum hydroxyls have been proposed with similar calculated activation energies (40 kcal/mol). However, the products in these cases have been computationally determined to be less stable that the reactant complex [14].
Hypoxanthine Oxidation Mechanism
The oxidation of hypoxanthine is theorized to occur much like the oxidation of xanthine. Once again the mechanism begins with the extraction of a proton from the hydroxyl of the molybdenum center by Glu1261. The deprotonated molybdenum oxygen is then free to engage in a nucleophilic attack on C2 of hypoxanthine paired with the concomitant hydride transfer from hypoxanthine to the molybdenum bound sulfur. The reactive species are stabilized by hydrogen bond interactions between the protonated Glu1261 and N2 of hypoxanthine as well as between Glu802 and N5 and the carbonyl of C4 of hypoxanthine. The subsequent electron transfer and product release occur similarly to the xanthine oxidation reaction [12].
Redox Potential
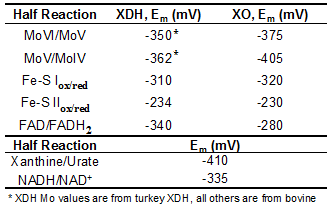
The redox potential of bovine XOR differs between the XO and XDH forms and may partly explain the difference in specificity for their final electron acceptors. Microcoulometry measurements of the mid-point potentials for the cofactors of XO at pH 7.7, 25 °C were as follows: MoVI/MoV, -375 mV; MoV/MoIV, -405 mV; Fe-S Iox/red, -320 mV; Fe-S IIox/red, -230 mV; FAD/FADH2, -280 mV [17]. The Em of xanthine/urate at pH 7.65 was -410 mV, indicating that the oxidation of xanthine by the enzyme is favored and that the electron affinity of the enzyme cofactors approximately follows FAD ≥ Fe/S > Mo [4]. In the case of XDH, the iron sulfur potentials appear similar to those of XO, - 310 and -234 mV for Fe-S I and Fe-S II respectively. However, the midpoint two electron reduction potential of FAD/FADH2 is -340 mV. The binding of NAD+ to the FAD domain increases this midpoint potential, facilitating the transfer of electrons from the iron-sulfur centers to FAD. Considering that the Em of NAD is -335 mV, the flavin midpoint potential of XDH appears suitable for the reduction of NAD+ to NADH while the corresponding potential FAD in XO (-280 mV) is too large [4]. A possible explanation for this shift in mid-point potential could be the exchange of interactions accompanying the conversion of XDH to XO. In XDH Asp429 is in direct interaction with the flavin coenzyme. However, the cleavage- or oxidation-induced shift of residues 422-432 exchanges Asp429 for Arg426, thereby modifying the electrostatic potential of FAD[2].
Links
2CKJ - Human milk XOR
1FO4 - Bovine xanthine dehydrogenase
1FIQ - Bovine xanthine oxidase
1WYG - Rat XDH triple cysteine mutant(C535A, C992R and C1324S)
2WE7 - XDH from Mycobacterium Smegmatis
1RM6 - 4-hydroxybenzoyl-CoA reductase (xanthine oxidase family)
1DGJ - Aldehyde Oxidoreductase
2CDU - NADPH oxidase from Lactobacillus sanfranciscensis
References
- ↑ Hille R. The Mononuclear Molybdenum Enzymes. Chem Rev. 1996 Nov 7;96(7):2757-2816. PMID:11848841
- ↑ 2.0 2.1 2.2 2.3 2.4 2.5 Enroth C, Eger BT, Okamoto K, Nishino T, Nishino T, Pai EF. Crystal structures of bovine milk xanthine dehydrogenase and xanthine oxidase: structure-based mechanism of conversion. Proc Natl Acad Sci U S A. 2000 Sep 26;97(20):10723-8. PMID:11005854
- ↑ 3.0 3.1 3.2 3.3 3.4 3.5 3.6 3.7 3.8 3.9 Nishino T, Okamoto K, Eger BT, Pai EF, Nishino T. Mammalian xanthine oxidoreductase - mechanism of transition from xanthine dehydrogenase to xanthine oxidase. FEBS J. 2008 Jul;275(13):3278-89. Epub 2008 May 30. PMID:18513323 doi:10.1111/j.1742-4658.2008.06489.x
- ↑ 4.0 4.1 4.2 4.3 Sanders SA, Massey V. The thermodynamics of xanthine oxidoreductase catalysis. Antioxid Redox Signal. 1999 Fall;1(3):371-9. PMID:11229448
- ↑ 5.0 5.1 5.2 5.3 5.4 Nishino T, Okamoto K, Kawaguchi Y, Hori H, Matsumura T, Eger BT, Pai EF, Nishino T. Mechanism of the conversion of xanthine dehydrogenase to xanthine oxidase: identification of the two cysteine disulfide bonds and crystal structure of a non-convertible rat liver xanthine dehydrogenase mutant. J Biol Chem. 2005 Jul 1;280(26):24888-94. Epub 2005 May 4. PMID:15878860 doi:10.1074/jbc.M501830200
- ↑ Vorbach C, Harrison R, Capecchi MR. Xanthine oxidoreductase is central to the evolution and function of the innate immune system. Trends Immunol. 2003 Sep;24(9):512-7. PMID:12967676
- ↑ McNally JS, Davis ME, Giddens DP, Saha A, Hwang J, Dikalov S, Jo H, Harrison DG. Role of xanthine oxidoreductase and NAD(P)H oxidase in endothelial superoxide production in response to oscillatory shear stress. Am J Physiol Heart Circ Physiol. 2003 Dec;285(6):H2290-7. Epub 2003 Sep 4. PMID:12958034 doi:http://dx.doi.org/10.1152/ajpheart.00515.2003
- ↑ Berry CE, Hare JM. Xanthine oxidoreductase and cardiovascular disease: molecular mechanisms and pathophysiological implications. J Physiol. 2004 Mar 16;555(Pt 3):589-606. Epub 2003 Dec 23. PMID:14694147 doi:10.1113/jphysiol.2003.055913
- ↑ Farquharson CA, Butler R, Hill A, Belch JJ, Struthers AD. Allopurinol improves endothelial dysfunction in chronic heart failure. Circulation. 2002 Jul 9;106(2):221-6. PMID:12105162
- ↑ 10.0 10.1 10.2 10.3 10.4 10.5 Pauff JM, Cao H, Hille R. Substrate Orientation and Catalysis at the Molybdenum Site in Xanthine Oxidase: CRYSTAL STRUCTURES IN COMPLEX WITH XANTHINE AND LUMAZINE. J Biol Chem. 2009 Mar 27;284(13):8760-7. Epub 2008 Dec 24. PMID:19109252 doi:10.1074/jbc.M804517200
- ↑ 11.0 11.1 11796116
- ↑ 12.0 12.1 12.2 12.3 12.4 Yamaguchi Y, Matsumura T, Ichida K, Okamoto K, Nishino T. Human xanthine oxidase changes its substrate specificity to aldehyde oxidase type upon mutation of amino acid residues in the active site: roles of active site residues in binding and activation of purine substrate. J Biochem. 2007 Apr;141(4):513-24. Epub 2007 Feb 14. PMID:17301077 doi:10.1093/jb/mvm053
- ↑ Leimkuhler S, Stockert AL, Igarashi K, Nishino T, Hille R. The role of active site glutamate residues in catalysis of Rhodobacter capsulatus xanthine dehydrogenase. J Biol Chem. 2004 Sep 24;279(39):40437-44. Epub 2004 Jul 20. PMID:15265866 doi:10.1074/jbc.M405778200
- ↑ 14.0 14.1 Amano T, Ochi N, Sato H, Sakaki S. Oxidation reaction by xanthine oxidase: theoretical study of reaction mechanism. J Am Chem Soc. 2007 Jul 4;129(26):8131-8. Epub 2007 Jun 12. PMID:17564439 doi:10.1021/ja068584d
- ↑ Okamoto K, Matsumoto K, Hille R, Eger BT, Pai EF, Nishino T. The crystal structure of xanthine oxidoreductase during catalysis: implications for reaction mechanism and enzyme inhibition. Proc Natl Acad Sci U S A. 2004 May 25;101(21):7931-6. Epub 2004 May 17. PMID:15148401 doi:10.1073/pnas.0400973101
- ↑ Choi EY, Stockert AL, Leimkuhler S, Hille R. Studies on the mechanism of action of xanthine oxidase. J Inorg Biochem. 2004 May;98(5):841-8. PMID:15134930 doi:10.1016/j.jinorgbio.2003.11.010
- ↑ Spence JT, Barber MJ, Siegel LM. Determination of the stoichiometry of electron uptake and the midpoint reduction potentials of milk xanthine oxidase at 25 degrees C by microcoulometry. Biochemistry. 1982 Mar 30;21(7):1656-61. PMID:6282314